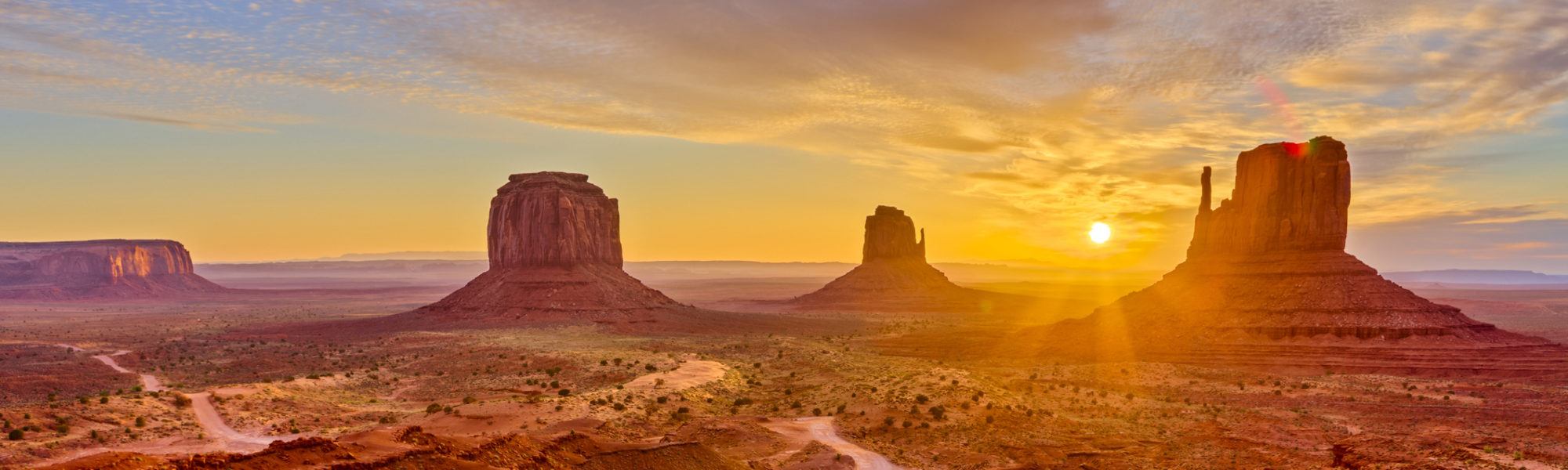
Modern Imaging Approaches In Neuroscientific Research*
Stephen L. Macknik, PhD
Division of Neurobiology, Barrow Neurological Institute, St. Joseph’s Hospital and Medical Center, Phoenix, Arizona
*Courtesy of Stephen L. Macknik, PhD
The Macknik Laboratory: Stephen Macknik received his PhD in neurobiology in the laboratory of Margaret Livingstone at Harvard University. He then worked at Harvard Medical School with David Hubel (Nobel Prize, 1981) on awake primate visual neurophysiology. He did a second postdoctorate in olfactory brain imaging with Zachary Mainen at Cold Spring Harbor Laboratory. Before joining the faculty of neurosurgery and neurobiology at Barrow, Dr. Macknik directed a laboratory in the Visual Science Department at University College London (UK). Shannon Bentz (research assistant) received his BA from Florida State University. He previously worked at the University of Maryland and Wake Forest University. Thomas Dyar (Senior Programmer/Data Analyst) received his BA from Boston University. Alex Schlegel (Programmer/Data Analyst, part-time) received his BS from North Carolina State University. He then worked at Dartmouth College and University College London.
Abstract
Brain mapping techniques have come a long way since the first explorations with surface electrodes. This study examines some of the new electrophysiological and imaging techniques that have been developed in response to the demands of brain mapping. Our laboratory combines several of these techniques, including single-unit recording, fMRI, optical imaging, and in vivo two-photon microscopy, to map cortical circuits with high-resolution and in three dimensions. Long-term goals of this research include the development of novel microscopic imaging methods to help diagnose and treat diseases of the human cerebral cortex.
Key Words: electrophysiology, fMRI, olfaction, optical imaging, single-unit, two-photon microscopy, vision
Abbreviations Used: BOLD, blood oxygenation level dependent; CT, computed tomography; EEG, electroencephalography; fMRI, functional magnetic resonance imaging; GFP, green fluorescent protein; PET, positron emission tomography; 2PLSM, two-photon laser scanning microscopy; SPECT, single photon emission computed tomography
Wilder Penfield, the neurosurgeon who founded the Montreal Neurological Institute, was not the only brain mapper of his time, but he was probably the most spectacular brain mapper of all time. Through mild cortical stimulation in locally anesthetized, fully aware neurosurgical patients, he discovered that he could evoke epileptic-like auras from his patients (which was his goal in order to localize epileptic foci). Moreover, he could evoke movements, feelings, and powerful episodic memories.[9] Most importantly, the same memory (or event) could be evoked repeatedly with repeated stimulation in the same location. Penfield thus showed that the cortex could be mapped for both sensory and cognitive functions, in an anatomically gross manner, by directly stimulating the brain with surface electrodes in awake patients, who themselves described the feelings and effects of the stimulation.
However, the spatial resolution of mapping across the surface of the brain was on the order of millimeters, whereas brain circuits are formed on the order of microns. The electrodes that Penfield used were essentially one-dimensional tools. Consequently, their ability to determine patterns of functionality (maps) within two-dimensional or threedimensional brain circuits was limited. This limitation is true in almost all electrophysiological approaches such as EEG, evoked potentials, and single- and multi-unit recordings.
This article describes several contemporary imaging techniques that can be combined for accurate assessment of cortical physiology in three dimensions associated with high spatial and temporal resolution. To understand the best use of the various imaging techniques, their deficiencies, and how they may best be combined, I will first review some functional anatomical techniques that predate contemporary imaging and that have laid the foundation for our current understanding of the brain.
Historical Perspective
David Hubel and Torsten Wiesel championed the combined use of single-unit recordings and anatomical staining techniques to achieve high-resolution cortical maps (see their new book for a historical review[4]). The tungsten micro- electrode, invented by Hubel,[3] was similar to the electrodes used by Penfield, but its tip was 100 to 1000 times smaller. Consequently, it could be used to discover meaningful, if limited (on the scale of tens of microns), functional patterns within brain areas. Hubel and Wiesel combined perpendicular, oblique, and tangential (to the cortical surface) metal electrode penetrations of the brain with histological staining techniques that reconstructed the electrode tracks in postmortem tissue. With these techniques they built the first crude model (on the scale of tens to hundreds of microns) of how neurons were arranged in a two-dimensional pattern across the surface of the primary visual cortex. They discerned functional circuits: repeating patterns of physiological domains across the surface of the cortex, such as ocular dominance, and stimulus orientation columns perpendicular to the surface of the cortex. They also determined general principles of structure and function of the cortical layers, which added a third dimension to their functional anatomical circuit model.
Ultimately, Hubel and Wiesel found that mapping the three-dimensional cortex with a one-dimensional device was too limiting. By combining their recordings with anatomical staining techniques, such as the Nauta method, radioactive 2-deoxyglucose uptake, horseradish peroxidase staining, and radioactive aminoacid pathway tracing, they were better able to correlate electrode tracks to direct postmortem imaging of cortical and subcortical maps.
Current Status
Hubel’s and Wiesel’s studies, which led to their Nobel Prize in 1981, spurred an explosion of functional anatomical studies in all brain systems (especially cortical systems) using similar techniques. The methods have been refined, but the combination of electrophysiological and anatomical approaches still remains the staple of cortical and subcortical brain mapping.
However, the status quo has notable disadvantages. First, the anatomy can only be visualized in postmortem tissue, which severely constrains its use both in cognitive research and in the clinic. In 1981 (and until recently), there was no method to obtain physiological information with high spatial and temporal resolution in vivo. Second, in 1981 the macroscopic maps on the surface of the cortex could not be visualized directly, in vivo. Finally, the above techniques were all highly invasive, with the exception of recording EEG or evoked potentials. The cognitive repertoires of scientists and neurosurgeons severely lacked a high-resolution brain-mapping technique that could be used in normal awake humans.
To overcome these deficiencies, several imaging techniques have since been developed (Table 1): fMRI, macroscopic optical recording, and 2PLSM. Other techniques (i.e., CT, PET, SPECT) are also extremely useful for specific but circumscribed uses in research and in the clinic. Consequently, the remainder of this article focuses on how fMRI, optical recording, and 2PLSM can be combined to explore cortical microcircuits and vascular function. No single technique is perfect, but fMRI, optical recording, and 2PLSM may be the best methods to combine to map circuits within the upper layers of cortex, in vivo.
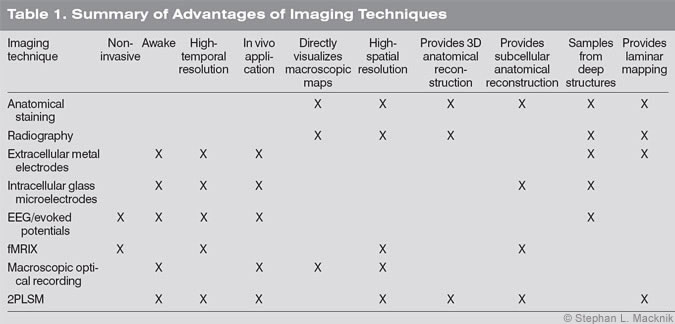
fMRI is the magnetic imaging of blood flow across different behavioral conditions. The magnetic properties of blood change as a function of neural activity (neural activity causes neurons to use more oxygen from the blood, and oxygenated blood has different magnetic properties than deoxygenated blood), making it particularly amenable to magnetic imaging analysis. Like iron, deoxygenated hemoglobin is magnetic whereas oxygenated hemoglobin (like rust) is not. By isolating the parts of the brain with deoxygenated blood from regions with oxygenated blood, we can infer where the neurons of the brain have absorbed the oxygen from the blood due to increased function. This reasoning led Ogawa and colleagues[8] to discover that BOLD signal can be used to map brain function noninvasively.
fMRI has sparked a major revolution in the discovery and crude mapping of cortical brain areas. However, the spatial resolution of the method is relatively low (on the order of millimeters rather than the micron resolution associated with anatomical staining techniques). Its temporal resolution is also low (the hemodynamic neural response occurs over seconds rather than the microsecond resolution associated with electrophysiology). Despite these shortcomings, fMRI is an excellent method for mapping many types of brain function and neuropathology, especially if further invasive scientific or neurosurgical interventions are planned.
We have used fMRI in collaboration with Peter Tse (Dartmouth College) to map visual brain areas noninvasively (Fig. 1) and also to map the parts of the visual system that may be relevant to maintaining visual awareness.[6] We are now extending this method to target brain areas for direct optical study.
Macroscopic Optical Imaging
Lack of oxygen not only changes hemoglobin from nonmagnetic to magnetic, it also turns it from reddish (rusty) to bluish (iron). Therefore, the parts of the brain’s surface that are active during a given task are bluer than the areas where neurons are less active. Gary Blasdel and Guy Salama[1] discovered that when red light was shone onto the exposed surface of the cortex, more red photons were absorbed by the active regions. The finding makes sense. In active regions, more oxygen was removed from the blood. Therefore, the blood turned bluish, which, by definition, means that it absorbs more red light.[7]
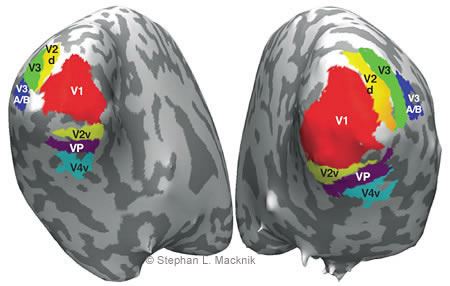
fMRI and macroscopic optical recording of blood flow have the same physical substrate (blood oxygenation level) and therefore the same slow temporal resolution. However, the spatial resolution of macroscopic optical recording is much higher than that of fMRI. The resolution of the capillary network immediately below the brain’s surface is tens to hundreds of microns, depending on the tissue.
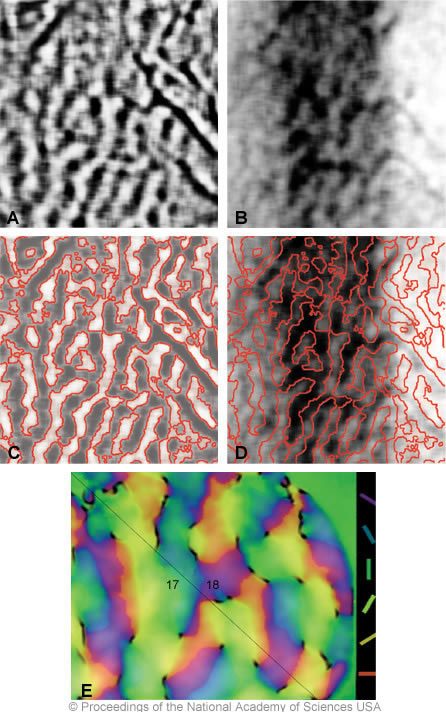
Figure 2. Macroscopic optical imaging of functional blood flow. (A) A 1 cm2 image from cortical area V1 in a primate. The stripes indicate an ocular dominance map created when visual stimuli are displayed to the right eye versus the left eye. (B) The same 1 cm2 of cortex when a flickering bar is displayed to one eye only. (C) Same image as in A, but with red lines describing the edges between the ocular dominance columns. (D) The red lines from C are overlapped on the image from B, revealing that the wispy white modula- tions of blood flow within the dark bar response are modulations caused by the response of the single eye to the bar. (E) An orientation map of the border between V1 and V2 from a cat (V1 and V2 are called area 17 and 18 in cats, by convention). The colors in- dicate stimulus orientation preference of the neurons in each point of the visual map. Figures 2A-D from Macknik SL and Haglund MM: Optical images of visible and invisible percepts in the primary cortex of primates. Proc Natl Acad Sci USA 96(26):15208-15210, 1999. “Copyright 1999 National Academy of Sciences, U.S.A.”
We have used optical recording in collaboration with Michael Haglund[5] (Fig. 2A-D) and Gary Blasdel (Fig. 2E) to analyze the functional anatomy of the early visual system. We have also done macroscopic optical imaging to target electrodes and 2PLSM in the olfactory system (Fig. 3).
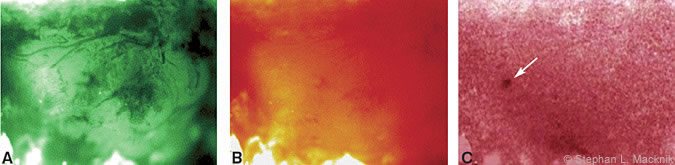
2PLSM
A major problem with standard light microscopy is that the light source is inevitably brighter than the tissue of interest, which drastically decreases the quality of the imaging. The images are also contaminated by light from sources outside the plane of focus. The invention of fluorescence microscopy was a major leap forward for microscopic analysis. It allowed tissue to be illuminated with one color of light (the excitation wavelength) while imaging only the fluoresced photons of a different color (the emission wavelength). Thus fluorescence microscopes filter photons outside the emission wavelength, thereby greatly improving the quality of the imaging. For biological imaging, the main problem with fluorescent imaging is that the quantum mechanical nature of fluorescence turns a high-energy (bluish) photon into a lower energy (reddish) photon. Because brain tissue tends to absorb blue photons, extremely high-powered light sources must be used to image tissues more than about 100 microns deep. Consequently, there is a risk of burning the brain instead of obtaining images.
In 1990 Denk, Strickler, and Webb[2] first used two-photon fluorescence excitation to overcome these problems. In the two-photon quantum effect, a fluorescent molecule with an excitation wavelength of 400 nm, for example, will fluoresce a photon when excited by two photons with wavelengths of 800 nm (each with exactly half the energy of a single 400-nm photon). Consequently, the same fluorescent dye will fluoresce a greenish emission photon after receiving a single bluish (400 nm) excitation photon or two simultaneous infrared (800 nm) photons.
The advantage is that the brain is relatively transparent to infrared light (compared with blue light) and one can image much deeper and with a much lower power light source (i.e., infrared) than when using a blue light source. Moreover, the illumination method is inherently focused on a given focal plane because the two infrared photons must land on the same fluorescent dye molecule within femtoseconds of each other to cause the emission of a green photon. Excitation (infrared) light scattered by irrelevant tissue outside the focal point causes no noise in the imaging (it is simply lost). Therefore, only the single point of focus (in three dimensions) of the laser will generate fluorescence. Because of these features, 2PLSM solves all of the earlier problems. It combines fluorescence (which allows noise to be filtered from the light source) with the ability to use an infrared light source to image deeply within cortex (on the order of 1 mm). Furthermore, its optical resolution is about the size of a single photon.
The remaining challenge to using two-photon imaging in physiological studies is to position the fluorescent dyes within live tissues. One way to address this issue is to use genetically modified animals that produce fluorescent molecules within the tissue of interest (e.g., by knocking-in genes taken from deep sea fish that glow). Special viruses that deliver fluorescence-inducing genes can also be injected into nongenetically modified tissue. In some cases, fluorescent dyes can simply be injected into the tissue of interest (the bloodstream or a neuron).
In collaboration with Zachary Mainen and Karel Svoboda at Cold Spring Harbor Laboratory, we used two-photon imaging to simultaneously image the neural structure of olfactory bulb glomeruli afferents and the capillary blood flow responses within the glomeruli. Using macroscopic optical imaging of blood flow, we first mapped the olfactory bulb glomeruli that responded to specific odorants (Fig. 3). We then injected red-colored fluorescein dextrans (red sugar) into the bloodstream and imaged the activated glomerulus using 2PLSM (Fig. 4). By capturing the red photons emitted with a red-filtered photomultiplier (photon detector), we recorded the velocity and volume of blood flow in rats and mice (Fig. 5).
Peter Mombaert’s laboratory at Columbia University created transgenic mice born with GFP already within the odorant receptor neurons of the olfactory system. Using these animals, we could simultaneously view the neural structure of the glomeruli using a greenfiltered photomultiplier. Consequently, we could determine exactly which capillaries inside the glomerulus had caused the macroscopic blood flow signal in Figure 3C. We then imaged the increase in blood flow caused within the relevant capillaries in response to olfactory activation. Future studies will focus on determining how activated neurons communicate that they need more oxygen to the microvasculature.
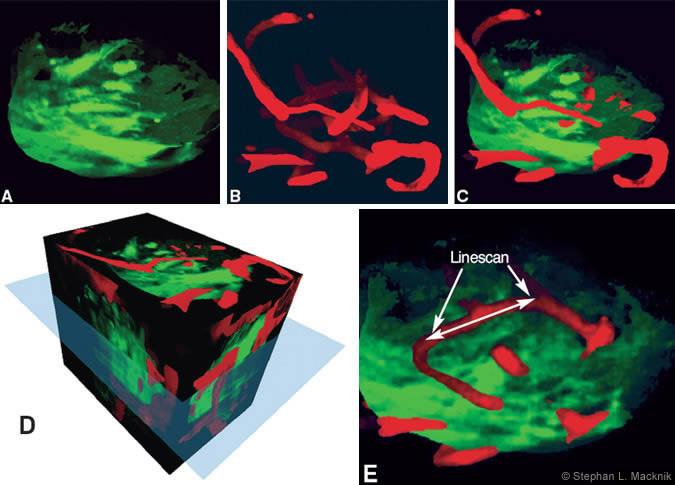
Future Directions
By combining the use of fMRI, macroscopic optical recording, and 2PLSM, we can target brain areas for study in awake behaving subjects (fMRI). We can then localize specific upper-layer cortical circuits during brain surgery (macroscopic optical recording). Finally, we can examine the specific targeted upper-layer circuits with high temporal and spatial resolution (2PLSM).
In the studies described here, we examined microvascular blood flow within specific functional neurocircuits. This work is particularly amenable to translation to the clinic because of its potential use in diagnosing microperfusion before and after microvascular neurosurgical interventions. These same techniques will help uncover how perfusion is controlled by the neural tissue. Moreover, it will provide neurosurgeons with the finest possible analysis of microvasular function before, during, and after vascular interventions. While fMRI and macroscopic optical recording are inherently methods for analyzing blood flow, 2PLSM can assess neuronal activity directly (after the neurons are filled with activity-dependent fluorescent dyes).
The combination of these techniques will no doubt lend itself to decades of discovery and microscopic diagnostics of cortical microperfusion and neural function. Nonetheless, new techniques still must be developed to examine deep brain structures in awake subjects to obtain the same spatial and temporal resolution now possible on the surface of the brain. Until deep imaging methods are developed, combined electrophysiological and postmortem anatomy techniques remain the only high-resolution source of determining functional anatomy in subcortical areas.
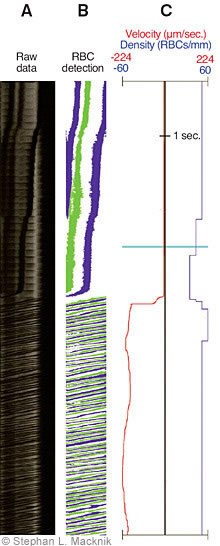
Figure 5. 2PLSM linescans of capillary blood flow. (A) Eight-second 2PLSM linescan of capillary denoted in Figure 4E. X-axis denotes spatial length of line- scan. Y-axis denotes time, with time increasing down- ward. Black lines indicate RBCs. (B) Results of custom RBC detection algorithm. Alternating RBCs are colored in green/blue. Stripe slope indicates velocity; number across X-axis indicates density. (C) Velocity (red line) and density (blue line) measurements through time (smoothed with 1-sec median filter). Cyan line indicates onset of hexanol odor. In this sample, ve- locity and density odor responses are not correlated.
References
- Blasdel GG, Salama G: Voltage-sensitive dyes reveal a modular organization in monkey striate cortex. Nature 321:579-585, 1986
- Denk W, Strickler JH, Webb WW: Two-photon laser scanning fluorescence microscopy. Science 248:73-76, 1990
- Hubel DH: Tungsten microelectrode for recording from single units. Science 156:549-550, 1957
- Hubel DH, Wiesel TN: Brain and Visual Perception: The Story of a 25-Year Collaboration. New York: Oxford University Press, 2005
- Macknik SL, Haglund MM: Optical images of visible and invisible percepts in the primary visual cortex of primates. Proc Natl Acad Sci U S A 96:15208-15210, 1999
- Macknik SL, Martinez-Conde S, Schlegel AA, et al: Awareness of monoptic and dichoptic visibility of simple targets is downstream of area V2, but processed within occipital cortex. Society for Neuroscience 34th Annual Meeting, San Diego, CA, October 23-27, 2004
- Malonek D, Grinvald A: Interactions between electrical activity and cortical microcirculation revealed by imaging spectroscopy: Implications for functional brain mapping. Science 272:551-554, 1996
- Ogawa S, Lee TM, Kay AR, et al: Brain magnetic resonance imaging with contrast dependent on blood oxygenation. Proc Natl Acad Sci U S A 87:9868-9872, 1990
- Penfield W, Japser H: Epilepsy and the Functional Anatomy of the Human Brain. Boston: Little, Brown, 1954