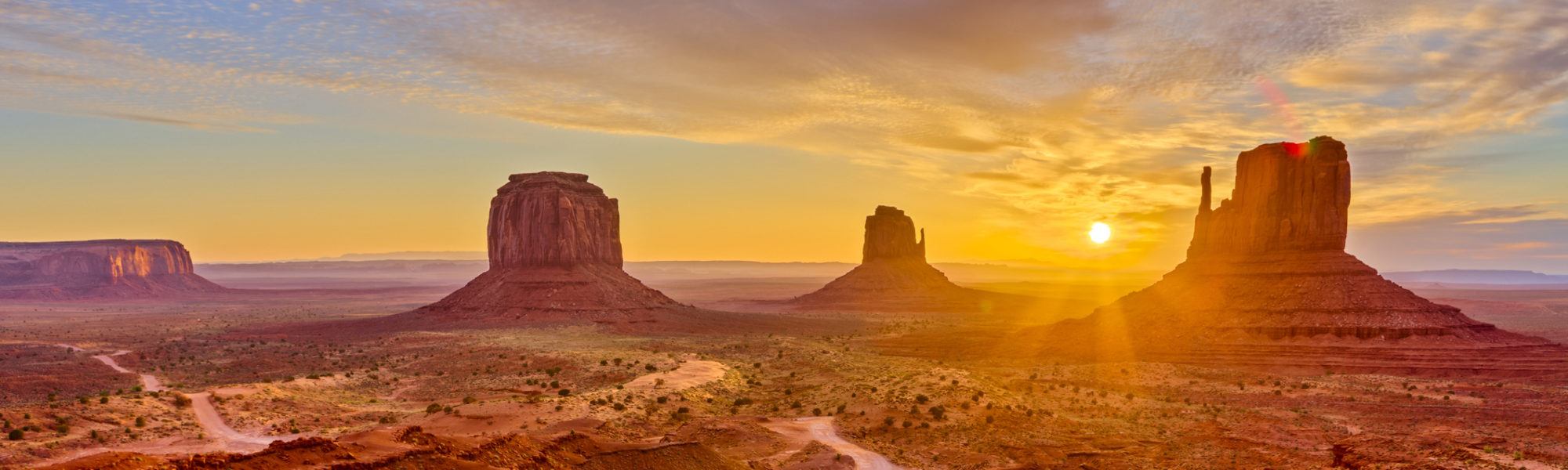
Spinal Mechanisms in the Generation and Control of Movement*
Thomas M. Hamm, PhD
Division of Neurobiology, Barrow Neurological Institute, St. Joseph’s Hospital and Medical Center, Phoenix, Arizona
*Courtesy of Thomas M. Hamm, PhD
The Hamm Laboratory: Tom Hamm received his PhD in physiology from the University of Tennessee, investigating the mechanical properties of skeletal muscle in the laboratory of Lloyd Partridge. He studied spinal motor function for several years with Douglas Stuart at the University of Arizona before joining Barrow Neurological Institute. Vladimir Turkin received his PhD from the Bogomolets Institute of Physiology in Kiev, Ukraine, analyzing auditory pathways of the cerebral cortex. He investigated the function of thalamic nuclei at Odessa State University before his arrival at Barrow. Derek O’Neill received a BS in zoology from Iowa State University. He was a supervisor in Laboratory Animal Care at Arizona State University before joining the Hamm Laboratory. Kalil Abdullah, a biotechnology student at Arizona State University, works part-time in the Hamm laboratory.
Abstract
Spinal nerve cells are a critical factor in the generation and control of movement. This article considers the contribution of spinal nerve cells to movement at several levels of complexity. These include the intrinsic properties of motoneurons and their modulation by other spinal neurons, reciprocal activation of motoneurons that innervate muscles with opposing actions, and the organization of activity in different muscles to produce complex movements like locomotion. Each of these topics is briefly discussed in relation to ongoing research.
Key Words: central pattern generation, dendrite, locomotion, motoneuron, Renshaw cell, spinal cord
Most of us think little about how we move and what is required to perform each movement that we make, whether reaching for an object, walking, playing tennis, or dancing. If we were to examine this process as an engineer would, we would be confronted with complicated sets of equations and puzzled by the many problems (some seemingly insurmountable) that our nervous system solves quickly. Much of the task of controlling movement is accomplished below the level of conscious awareness, using networks and nerve cells of the cerebellum, brainstem, and spinal cord. In our laboratory we seek to understand the role of spinal neurons and networks in the process by which complicated patterns of muscle activity are created to perform the varied and complex movements of which we are capable. Knowledge and understanding of these neurons are also needed to develop treatments for repair and therapy after spinal injury and other injuries and diseases that result in dysfunction of the spinal cord.
Spinal Motoneurons
The most intensively studied spinal neurons are motoneurons, which form the final pathway from the nervous system to muscles. In the human spinal cord, about 60,000 motoneurons innervate the muscles of each leg.[18] Each motoneuron innervates a set of muscle fibers, each member of the set possessing the same metabolic, biochemical, and physiological properties.[9,14] These properties endow each motor unit (a motoneuron and the muscle fibers it innervates) with a capability adapted for particular types of activity, such as maintained activity at low forces, as needed for walking, distance running, and other aerobic activities, or brief, explosive movements that produce large forces for brief periods, as needed for sprinting and weight lifting. The complement of motor unit characteristics varies between muscles and from one individual to the next, depending on genetics, training, or patterns of use.[7] The activity of each motoneuron determines the force produced by its innervated muscle fibers. The set of motoneurons selected for activation determines the characteristics of the contraction and the pattern of torques produced and, in interaction with the mechanics of the body and the environment, the movement an individual achieves. Within the group of motoneurons that innervate each muscle, the motoneuron pool, motoneurons normally are activated in an orderly sequence according to their size. Each larger motoneuron tends to activate a set of muscle fibers that produces a larger force than the motoneuron just activated.[3]
To understand how the contraction is produced, how movement is controlled, and how control can become disordered after disease and injury, we must first understand how the motoneuron is activated and identify and understand the factors that determine its activity. We also must determine how spinal neurons, interacting with the descending nerve fibers of supraspinal neurons and of peripheral sensory and reflex pathways, generate the temporal patterns and combinations of muscle activity that yield well-controlled, purposeful movements.
The motoneuron is a cell of considerable complexity. One of the largest nerve cells in the nervous system, each motoneuron has 6 to 18 stem dendrites. Each dendrite branches repeatedly to form an extensive, complex dendritic arbor consisting of hundreds of segments that extend 3 to 4 mm across the spinal cord.[19] This complex structure provides a huge area to receive synaptic inputs from sensory, supraspinal, and other spinal neurons.
It was long thought that integration of the various inputs to motoneurons was a passive process. The simple electrical properties of the motoneuron (the resistance and capacitance of its dendritic membranes and its intracellular resistance) were thought to determine how synaptic currents depolarized the motoneuron to a threshold voltage, inducing action potentials and contraction of the motor unit. However, this concept of the motoneuron was inadequate. The measured strengths of synaptic inputs from sensory fibers and other spinal neurons are sufficient to produce only low levels of activity compared to the full functional range of motoneurons.[3] Studies showing that motoneurons have a complement of conductance channels sensitive to membrane voltage and that some of these conductance channels make an important contribution to the excitability of motoneurons at and below the threshold for action potentials promise to resolve this difficulty.[1,2,15,16]
The intrinsic currents generated by these voltage-activated conductance channels can amplify the synaptic currents generated by sensory and spinal neurons so that these inputs can exert significant effects on motoneuron activity. A key problem now is to determine how intrinsic and synaptic currents interact within motoneurons, requiring knowledge of their relative strengths and locations within the dendritic arbor. Moreover, knowledge of how these factors change after injury or disease may provide insight into the nature of motor dysfunction in such disorders and suggest treatments.
We have addressed this problem by developing a test that uses measurements of electrical impedance to determine the location and size of conductances in dendrites produced by synaptic inputs or intrinsic, voltage-dependent ion channels.[13] Electrical impedance is the ratio of voltage to current, in this case the membrane voltage produced by injection of current into the soma of a motoneuron of a laboratory animal. Impedance is a function of the frequency of the injected current. It has a distinctive profile depending on the passive and voltage-dependent properties of the motoneuron and on its structure.
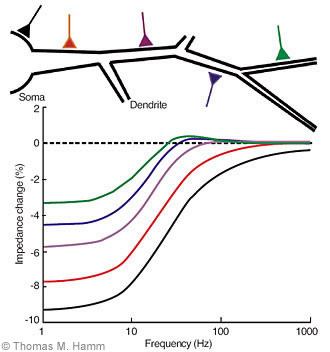
During activation of a voltage-dependent or synaptic conductance, the impedance of a motoneuron changes. This change has a distinctive form that depends on the location of the conductance and on its magnitude (Fig. 1). The range of frequencies over which synaptic conductance causes a decrease in impedance increases the closer the conductance is to the soma of the neuron. The magnitude of the conductance determines the size of the decrease in impedance at these frequencies. By determining the frequency range and the size of the change in impedance, the location and magnitude of the conductance can be estimated.
By collecting this information for different conductances, how different synaptic inputs interact with each other and the motoneuron’s own conductances to control its activity can be analyzed. We recently identified the location and conductance of synapses on motoneurons from Renshaw cells.[12] These spinal neurons provide feedback about motoneuron activity and regulate the activity of motoneurons and another type of spinal neuron. (The Renshaw cell was named after its discoverer, Birdsey Renshaw, whose promising career was cut short by his death from polio.)
The voltage developed in a motoneuron dendrite by application of an excitatory synaptic current in the dendrites and the effect on this voltage by interaction with intrinsic currents and the inhibitory current produced by Renshaw cells can be simulated (Fig. 2). At increasing values of excitatory conductance, the depolarizing voltage increases. With sufficient depolarization, the intrinsic conductance of the dendrite is activated, further depolarizing the dendrite and amplifying the excitatory effect of the synaptic input. The excitatory input needed to produce this effect is increased substantially by modest amounts of inhibitory conductance produced by the Renshaw cells. Such modulation arising from interactions among several dendritic conductances is an important determinant of the activity of motoneurons and of an individual’s capacity to produce and control movement. We hope that their investigation by this approach will enrich our understanding of their function in health, disease, and injury.
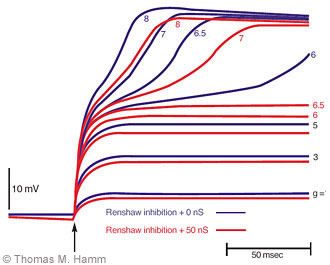
Reciprocal Activation
To produce most movements, the activities of motoneurons innervating many muscles must be coordinated. Understanding the process by which this coordination is achieved is one of the great challenges in motor research. One of the simplest forms of coordination is the reciprocal activation of antagonist muscles, those whose mechanical actions oppose each other. Alternating movements, like those performed in walking, are characterized by the coordinated contraction of one muscle while its antagonist relaxes. In simpler vertebrates (fish, reptiles and amphibians),[4-6,17] such reciprocally organized activity is produced by a simple spinal mechanism. The neurons that provide synaptic excitation to activate one set of motoneurons also excite neurons that inhibit the motoneurons of the antagonist muscle, causing that muscle to relax as its opponent contracts (Fig. 3).
Is the same mechanism used in the mammalian spinal cord? The question is important because the reciprocal activation that underlies the ability to perform normal movements is often disrupted after spinal injury and other nervous system disorders. Reciprocal activation can occur in spinal-injured subjects after they have been trained to walk on a treadmill.[11] This process relies on spinal nerve cells, even though reciprocal activation remains impaired in other voluntary movements. Knowledge of the spinal mechanisms of reciprocal activation is needed to develop means to restore this ability when it is lost to injury and disease.
Our investigations show that the mammalian spinal cord uses the same mechanism as used by other vertebrates to produce reciprocal activation during rhythmic movements like walking and scratching. In experimental work with laboratory animals, spinal neurons can be activated to generate the rhythmic patterns of neural activity responsible for such movements while recordings are made from motoneurons and muscle nerves. Intracellular recordings of the voltages in motoneurons show alternating cycles of depolarization and hyperpolarization that excite the motoneurons and inhibit them, respectively. The cycles of depolarization coincide with bursts of activity in other flexor motoneurons, while extensor motoneurons discharge during the periods of hyperpolarization (Fig. 3).
Occasionally, such patterns show irregularities in which one cycle of activity in a muscle nerve is lost (Fig. 3), even though the nerve to the antagonist muscle continues to discharge rhythmically. When this irregularity occurs, motoneurons to the antagonist muscle lose a cycle of hyperpolarization, while continuing to receive rhythmic waves of excitation. Moreover, the amplitudes of the hyperpolarizing potentials are correlated with the activity of opposing muscle nerves in normal cycles. Thus inhibition of motoneurons depends on the excitation of their opponents as expected from the simple mechanism just discussed.
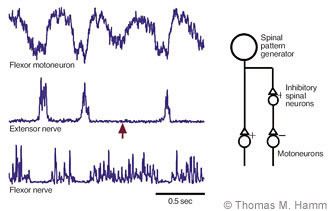
Which spinal neurons mediate this inhibition? An identified group of neurons that mediates reciprocal inhibition during simple reflexes is the primary candidate, although some evidence is inconsistent with this proposal. Comparison of the dendritic locations of synapses from these neurons with those of synapses that produce rhythmic inhibition may answer this question.
Organization of Spinal Neurons
Coordination also requires the proper timing and balance of activity between motoneurons and muscles that are coactive during movements. As noted, the spinal cord has the capacity to generate the complex, coordinated patterns of activity for walking and other rhythmic movements. How the spinal cord generates and controls these patterns remains a central problem in the control of movement. Are spinal neurons organized to control different sets of motoneurons whose muscles have distinct mechanical functions (e.g., hip extension vs. ankle extension)? Does the spinal cord generate a common command that coactivates all muscles needed at each moment in the task? Does the spinal cord generate several commands that can be combined to produce the appropriate balance of muscle activities? Knowledge of the mechanisms by which the spinal cord performs these tasks, of how they can become disordered after disease and injury, and of their capacity for recovery with training and other therapeutic procedures is critical for devising new treatments for restoration of function.
We have investigated the process by which the spinal cord generates rhythmic movements by an indirect approach, examining the activity in motoneurons and muscle nerves for information about the organization of the spinal neurons responsible for the rhythmic patterns of activity. During such activities, motoneurons receive a barrage of synaptic inputs that elicits trains of action potentials from each active motoneuron. Synaptic inputs can produce correlations in the synaptic currents and activity of the motoneurons that receive these inputs in common. The presence of such correlations during the generation of rhythmic motor patterns indicates that motoneurons receive similar rhythmic synaptic drives. By identifying which motoneuron pools have correlated synaptic potentials and activities, we can gain insights into the organization of the spinal neurons that generate rhythmic motor activities.[8]
We have examined the synaptic potentials in individual motoneurons and the activity of many motoneurons (in muscle-nerve recordings) to determine which motoneuron pools have correlated activity and synaptic potentials and to determine how spinal neurons are organized to activate the motoneurons of different muscles during walking and other rhythmic actions. These studies show patterns of correlations between different sets of motoneuron pools.[10]
A typical finding (Fig. 4) is a correlation between the synaptic potentials of a motoneuron and the activity recorded from a muscle nerve that innervates a muscle with a distinctly different mechanical action than the muscle innervated by the motoneuron. A coherence function shows the correlation (normalized to a maximum value of 1) as a function of the frequency of the recorded signals. Significant correlations (segments in which coherence exceeds the dashed 99% confidence limits) typically occur at frequencies that have been observed in the discharge of spinal neurons during rhythmic activity.
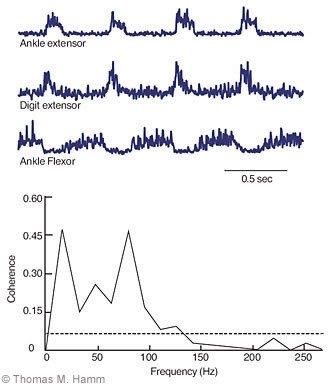
Such correlations could be produced by the actions of neurons whose axons project to each of the correlated motoneuron pools. Alternatively, they could arise from the actions on motoneurons of distinct sets of the spinal neurons whose activities are themselves correlated. In either case, these results indicate that spinal neurons provide an important component of the coordination required between muscles that act at different joints needed to perform many activities. By the use of this correlational approach and the other methods described in this article, we hope to develop a better understanding of how this coordination is achieved.
Acknowledgments
These studies have been supported by NIH grant NS22454 and by funds from the Barrow Neurological Foundation and the Barrow Women’s Board.
References
- Bennett DJ, Hultborn H, Fedirchuk B, et al: Synaptic activation of plateaus in hindlimb motoneurons of decerebrate cats. J Neurophysiol 80:2023-2037, 1998
- Binder MD, Heckman CJ, Powers RK: How different afferent inputs control motoneuron discharge and the output of the motoneuron pool. Curr Opin Neurobiol 3:1028-1034, 1993
- Binder MD, Heckman CJ, Powers RK: The physiological control of motoneuron activity, in Rowell LB, Shepherd JT (eds): Handbook of Physiology. Exercise: Regulation and Integration of Multiple Systems. New York: Oxford University Press, 1996, pp 3-53
- Buchanan JT, Grillner S, Cullheim S, et al: Identification of excitatory interneurons contributing to generation of locomotion in lamprey: Structure, pharmacology, and function. J Neurophysiol 62:59-69, 1989
- Dale N: Reciprocal inhibitory interneurones in the Xenopus embryo spinal cord. J Physiol 363:61-70, 1985
- Dale N, Roberts A: Dual-component amino-acidmediated synaptic potentials: Excitatory drive for swimming in Xenopus embryos. J Physiol 363:35-59, 1985
- Edgerton VR, Bodine-Folwer SC, Roy RR, et al: Neuromuscular adaptation, in Rowell LB, Shepherd JT (eds): Handbook of Physiology. Exercise: Regulation and Integration of Multiple Systems. New York: Oxford University Press, 1986, pp 54-88
- Hamm TM, McCurdy ML, Trank TV, et al: The use of correlational methods to investigate the organization of spinal networks for pattern generation, in Cope TC (ed): Motor Neurobiology of the Spinal Cord. Boca Raton, FL: CRC Press, 2001, pp 135-170
- Hamm TM, Nemeth PM, Solanki L, et al: Association between biochemical and physiological properties in single motor units. Muscle Nerve 11:245-254, 1988
- Hamm TM, Trank TV, Turkin VV: Correlations between neurograms and locomotor drive potentials in motoneurons during fictive locomotion: Implications for the organization of locomotor commands. Prog Brain Res 123:331-339, 1999
- Maegele M, Müller S, Wernig A, et al: Recruitment of spinal motor pools during voluntary movements versus stepping after human spinal cord injury. J Neurotrauma 19:1217-1229, 2002
- Maltenfort MG, McCurdy ML, Phillips CA, et al: Location and magnitude of conductance changes produced by Renshaw recurrent inhibition in spinal motoneurons. J Neurophysiol 92:1417-1432, 2004
- Maltenfort MG, Phillips CA, McCurdy ML, et al: Determination of the location and magnitude of synaptic conductance changes in spinal motoneurons by impedance measurements. J Neurophysiol 92:1400-1416, 2004
- Nemeth PM, Solanki L, Gordon DA, et al: Uniformity of metabolic enzymes within individual motor units. J Neurosci 6:892-898, 1986
- Prather JF, Powers RK, Cope TC: Amplification and linear summation of synaptic effects on motoneuron firing rate. J Neurophysiol 85:43-53, 2001
- Rekling JC, Funk GD, Bayliss DA, et al: Synaptic control of motoneuronal excitability. Physiol Rev 80:767-852, 2000
- Robertson GA, Stein PS: Synaptic control of hindlimb motoneurones during three forms of the fictive scratch reflex in the turtle. J Physiol 404:101-128, 1988
- Tomlinson BE, Irving D: The numbers of limb motor neurons in the human lumbosacral cord throughout life. J Neurol Sci 34:213-219, 1977
- Ulfhake B, Kellerth JO: A quantitative morphological study of HRP-labelled cat alpha-motoneurones supplying different hindlimb muscles. Brain Res 264:1-19, 1983